V.
Taking Flight: Post-Retinal Processing
Collo- & Lemno-Thalamic
Visual Pathways
Amniote visual
pathways. The two major visual pathways found
in reptiles, birds, and mammals show a high degree of anatomical similarity.
These two pathways arise from retinal efferents which travel to either
the optic tectum (TeO in birds, superior colliculus in primates) or dorsal
thalamus (OPT in birds, LGNd in primates). The retino-collicular
pathway has been called a tectofugal (Karten & Revzin, 1966)
or collothalamic pathway (Butler & Hodos, 1996). From
the collicular structure there are efferents to the thalamus (nucleus Rotundus,
Rt, in birds, LP/pulvinar in primates). The thalamic nuclei send
projections to the telencephalon (ectostriatum in birds, extrastriate cortex
in primates). Other retinal efferents travel to dorsal thalamic nuclei
travel to areas in the telencephalon (visual wulst in birds, striate cortex
in primates). This retino-thalamo-striate pathway has been called
a thalamofugal (Karten & Nauta, 1968) or lemnothalamic pathway
(Butler & Hodos, 1996).
While there are many similarities in
the avian and mammalian visual pathways, the relative importance of each
pathway to visual perception appears to be different. The past
three decades of work on mammalian visual systems has concentrated on the
geniculo-striate system. Studies on the lateral geniculate nucleus
of the dorsal thalamus, stiate cortex (V1), and extrastriate areas (e.g.,
V4, MT) have provided a wealth of detail on the visual systems of mammals
and how brains perform visual processing in general. In birds, however,
the situation is somewhat reversed. The avian collothalamic system
may be the dominant visual pathway, serving many of the functions ascribed
to the primate lemnothalamic system. This generalization appears
to be true in at least lateral-eyed species like the pigeon and zebra finch.
Lemnothalamic
pathways & binocular vision. Binocular vision presumably evolved
independently in birds and mammals, given the differences in their retinal
decussation patterns. The pattern of optic nerve decussation
in birds is very different from that of the primates. Decussation
patterns relate to the extent to which the eyes are more frontally or laterally
placed in the skull, and hence the degree of binocular processing that
placement allows. Most birds and reptiles have laterally-placed eyes
and possess full decussation, whereby the optic nerves from both
nasal and temporal hemiretina project to the contralateral side of the
brain. Primates have a partial decussation pattern, whereby
only the optic nerve fibers from the nasal hemiretina cross to the contralateral
side while those of the temporal hemiretina project ipsilaterally.
Partial decussation is found in most mammals, with the degree of uncrossed
fibers varying from 10% in rabbit (who once were thought to lack any binocular
vision) to 50% in non-human primates and man (Pettigrew, 1991). The
existence of some intermediate evolutionary step whereby both partial
decussation at the optic chiasm and a bilateral projection from the thalamus
existed is unlikely if topographic relations were to be maintained in such
an organism.
Organisms exhibiting full decussation
of the optic nerves from the retina may still possess binocular vision.
It was traditionally thought that partial decussation was essential for
binocular vision, since it allows information from both eyes to converge
on the same brain structures. This, however, does not appear to be
true due to a unique arrangement of visual projections in some birds known
as a double decussation. Predatory birds, such as owls and
some diurnal raptors, tend to be those with more frontally-placed eyes
and presumably larger binocular visual fields used for stereoscopic vision.
In owls, despite the initial full decussation of the optic nerves, a recrossing
occurs via a second decussation from the principle thalamic optic nucleus
(OPT, also called the dorsal lateral geniculate nucleus, GLd). The
fibers of the second decussation are those originating from the temporal
retina, which are most involved in binocular processing (Pettigrew, 1991).
Visual thalamic neurons then project bilaterally to visual wulst (e.g.,
in owls, kingfishers, some nightjars, and diurnal birds of prey) where
binocular integration occurs (Pettigrew, 1991).
In birds there is a positive correlation
between the extent of the lemnothalamic pathway and the development of
binocular vision. Owls have an extensively developed OPT and
visual wulst (Karten et al., 1973), and binocular processing has been demonstated
in owls' wulst (Pettigrew, 1979). Lateral-eyed birds like the pigeon
have a less extensive wulst and smaller binocular fields. In pigeons,
OPT receives retinal input mostly from the region for lateral viewing before
sending projections to visual wulst (Güntürkün et al., 1993).
Binocular processing could still occur in the pigeon's lenmothalamic pathway,
since descending projections from wulst terminate in the OPT, which then
sends bilateral projections to the wulst (Güntürkün et al.,
1993). However, it is possible that collothalamic "dominance" (at
least in pigeons) may relate more to feeding strategies than having lateral
eyes. A diurnal, lateral-eyed raptor, the kestral (Falco sparverius)
has an over-representation of its frontal binocular visual field in the
lemnothalamic pathway (Pettigrew, 1978).
The importance of the lemnothalamic
visual system in primates may relate to nocturnal habits early in mammalian
evolution. The collothalamic system of the early mammals probably
remained relatively stable during their "nocturnal" period (see Bottleneck
Theory, this chapter). The topographic mapping of the optic tectum
and collothalamic visual pathways should have been sufficient to serve
their needs in preying on insects or perhaps small reptiles. Other
parts of the brain, including the olfactory and auditory systems may have
continued to rapidly evolve. The olfactory system already present
in the mammal-like therapsid reptiles retained its importance as a means
to locate prey and perhaps conspecifics for reproduction. The auditory
system, in conjunction with the rod dominated retina, could have become
increasingly sophisticated, enabling efficient localization of prey under
scotopic conditions. The spectacular growth of the cerebral cortex
may also have begun during this period. This may also be the
point in mammalian evolution when the importance of the lemnothalamic visual
system took hold. Connections from the retinal ganglion cells to
the dorsal thalamus (LGNd in primates) were added to existing retino-collicular
connections. These retino-geniculate connections then formed increasingly
complex connections with striate cortex. These connections gained
in importance in serving visual functions, functions which seem to be served
by collothalamic systems in birds and reptiles.
Support for the expansion of lemnothalamic
function comes from the study of modern snakes. Snakes are presumed
to have evolved from lizards which began to live a burrowing existence
and eventually "lost" their limbs. The sensory systems of snakes
appear to have been reduced during this burrowing period. Upon re-attaining
niches in the water and above ground, snakes appeared to have "rebuilt"
their visual systems with a reliance on retino-geniculate connections.
The optic tectum and nucleus rotundus (collothalamic components) in snakes
(e.g., the water snake Natrix) are reduced compared to other reptiles;
however, the dorsal lateral geniculate nucleus is relatively large (Northcutt
& Butler, 1974). Since portions of the lemnothalamic pathways
of snakes are more developed than in other reptiles, it appears that upon
re-emergence from their burrowing existence, it was the lemnothalamic system
which continued to evolve to serve visual functions. Perhaps the
collothalamic system was too reduced, or not neurologically plastic enough,
to serve the visual processing roles which became the domain of the lemnothalamic
visual pathways.
The lemnothalamic, or geniculo-striate,
system of primates is the most developed of the mammals. In primates,
approximately 90% of the retinal ganglion cells send projections to the
lateral geniculate nucleus of the dorsal thalamus (LGNd), with the remainder
projecting to the pretectum and superior colliculus. Damage to the
striate cortex (V1) in humans can lead to a condition known as blindsight,
in which the patient is not consciously aware, but can locate a moving
object in the affected visual hemifield. This spared ability in the
"blind" area is presumably the result of the processing ability of the
collothalamic visual system. In addition, the extrastriate cortex
is the sight of numerous important visually-responsive areas involved in
color (V4), form (IT), and motion perception (MT).
The reliance of primates on binocular
vision and stereopsis may have driven the development of the lemnothalamic
visual system. It appears
that all mammals have some degree of binocular vision. Binocular
vision was probably present in the earliest mammals, and contributed to
their success as a class. Stereopsis increases the signal to noise
ratio in low light conditions, serving as a camouflage breaker.
In addition, the conjugate eye movements of mammals to fixate objects of
attention on the area centralis or fovea set mammals apart (Walls, 1942).
Frontal vision has also led to an increased ganglion cell density in frontally
directed retina, enhancing visual acuity. These traits are exceptionally
developed in the primates. Early fossil primates show large, frontally
placed eyes and it is quite reasonable to assume they had binocular visual
systems as good as those of modern prosimian primates (Pettigrew, 1991).
The binocular field is probably also related to the praxic field (i.e.,
the use of visually-controlled activity to manipulate objects). The
ability of the primates and their predecessors to use tools may have contributed
to lemnothalamic development, as well as refinements in motor control and
increases in poly-sensory cortical areas. Binocular vision,
stereopsis, and tool use must have had an extraordinary effect on visual
evolution in primates.
Collothalamic
pathways in lateral-eyed birds. Evidence suggests that the lemnothalamic
pathway is not as important to visual processing in lateral-eyed birds
as it is in mammals. The avian lemnothalamic pathway is comparable
to the geniculo-striate mammalian pathway. The visual wulst has both
anatomical and physiological similarities to mammalian striate cortex including;
laminar appearance, receipt of dorsal thalamic efferents, and cells characterized
by small receptive fields. The contribution
of the lemnothalamic pathway to visual perception in the pigeon is currently
unclear. Neurobehavioral studies show surprisingly mild deficits
in visual discrimination performance after lesions of the pigeon OPT and
visual wulst in lateral-eyed birds. Numerous studies employing discriminations
of intensity, pattern, and color yield either no discernible deficits or
only mild to moderate ones (Hodos et al., 1973; Hodos & Bonbright,
1974; Delius et al., 1984; Chaves et al., 1993). Thus, it appears
the lemnothalamic pathway is not crucial to these primary visual functions,
and that the collothalamic pathway can perform simple visual discriminations
without lemnothalamic processing. It has been suggested, however,
that the traditional means of testing visual discriminations with projection
of the stimulus onto the response keys may affect the outcomes of these
studies. The birds are required to fixate the test stimuli with their
frontal visual fields (served presumably by the tectofugal pathway), and
therefore lesions of OPT or visual wulst would show little effect.
Hahmann and Güntürkün (1993) demonstrated that visual acuity
of head-fixed pigeons with OPT lesions was unimpaired for frontal acuity,
but subjects suffered performance deficits even for course grating patterns
presented to the lateral fields.
The collothalamic pathway appears to
dominate visual functions in pigeons, and perhaps most lateral-eyed birds.
Collothalamic pathway lesions result in severe deficits in various visual
discrimination tasks with brightness, color, and patterns (Hodos, 1976;
Engelage & Bischof, 1993). Such lesions can also result in significant
reductions in acuity, and in thresholds for discriminating brightness and
size (Hodos, Macko, & Bessette, 1984; Hodos, Weiss, & Bessette
1986, 1988). In particular, lesions of Rt and ectostriatum
can have profound effects on visual discrimination. Lesions of Rt
have severe, detrimental effects on intensity, pattern, and color discrimination
tasks (Hodos, 1969; Hodos & Karten, 1966). 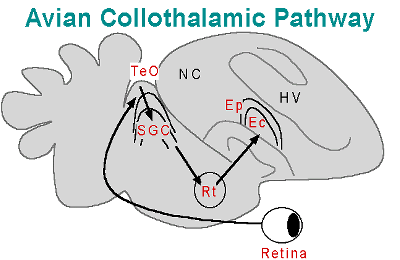
Lesions in the ectostriatum
cause severe, and sometimes irreversible, deficits in visual performance
for brightness, color, and pattern discriminations (Hodos et al., 1973;
Hodos et al., 1984; Bessette & Hodos, 1989). Ectostriatal lesions
also affect the ability of pigeons to visually distinguish between natural
concepts (e.g., food versus non-food); (Watanabe, 1991). Lesions which involve both the lemnothalamic
and the collothalamic systems result in severe deficits in discriminations
of a variety of stimuli. Such lesions produce deficits that are greater
than when either pathway alone is disrupted (Hodos & Bonbright, 1974,
Hodos et al., 1973; Macko & Hodos, 1984; Riley et al., 1988).
Combined lesions of the lemno- and collothalamic systems are particularly
detrimental to spatial resolution tasks, producing greater deficits than
birds with only collothalamic lesions (Macko & Hodos, 1984; Hodos et
al., 1984).
.The
Flying Brain
Powered
flight and motion processing. The development of powered flight and
the complexity of visual analysis it requires gradually changed the reptilian
brain of avian ancestors to the form seen in modern birds. Active
flight requires a very high output of power (work per unit time), but is
more efficient than running (but less efficient than swimming) in terms
of the transport of a unit mass over a unit distance (Norber, 1985).
The demands of flight have had a tremendous impact on birds' body size,
weight, skeletal morphology, feather shape and number, feeding styles,
brain size, et cetera. Powered flight has also contributed
to the tremendous diversity and number of bird species, enabling them to
cross natural barriers and fill almost every ecological niche on earth.
Flight requires a more specialized visual apparatus than that which birds
inherited from their reptilian forebears.
The difference between human and avian
velocity thresholds may indicate that the visual system of birds is biased
toward the recognition and calculations of higher velocity targets.
Thresholds for detection of velocity in pigeons, as determined by Hodos
and colleagues (1975) indicated retinal velocity ranges of 4.1-6.01 deg/sec;
human observers can detect retinal velocities of 3min/sec (Graham, 1968),
roughly .05deg/sec. Studies in our lab indicate that birds have more
difficulty assessing the direction of moving dots on a stimulus display
for "slow" speeds (16.63 cm/sec) than "fast" speeds (3.34 cm/sec); (Laverghetta
and Shimizu, 1999). On the other hand, the velocity detection data
for pigeons may be underestimated, given the fact that the subjects were
tested with stimuli presented in their frontal fields. Birds (at
least some species) appear to use frontal viewing for stationary stimuli
but orient their lateral visual fields when viewing fast moving stimuli
(Maldonado et al., 1988).
The high proportion of directionally-selective
and motion-responsive units in the collothalamic visual pathways, compared
to that of reptiles, illustrates adaptations required for an air-borne
lifestyle. The collothalamic visual pathway in birds exhibits high
proportions of motion and directional responses at the mesencephalic, diencephalic,
and telencephalic levels. Birds, with the possible exception of the diurnal
primates (e.g., humans), are the vertebrates which may rely most heavily
on vision to function in their environment. The most obvious visually-dependent
behavior of birds is flight. The navigation of three dimensions where
X, Y, and Z plays a role in the approach or avoidance of stimuli in the
environment requires a high degree of visual processing.
Birds also exhibit an impressive range
of visually guided behaviors other than flight. An aerial lifestyle
also requires different breeding and feeding strategies. Feeding
is also dependent on visual cues in most avian species, whether a predatory
raptor or a grainiverous ground-feeder. Sophisticated visual abilities
have allowed the development of good recognition of predators and conspecific
mates or rivals. These abilities have, in turn, expanded the behavioral
repertoire of birds; in many species it has enabled courtship displays
and the formation of pair-bonding and social hierarchies. Given the
various specializations driven by a species' individual ecology, the following
ideas apply generally to most birds. Birds brains, deriving from
the basic reptilian plan, maintained a prominent collothalamic visual system.
This system became even more specialized for the demands of flight, and
came to serve sophisticated visual abilities used in sexual selection,
nesting, territoriality, and conspecific recognition.
The Brains of Pterosaurs
and Birds
Pterosaurs
- optic lobes. Comparing pterosaur brains with those
of other reptiles and modern birds illuminates what its structure contributed
to flight mechanisms, and perhaps other parts of its lifestyle which it
did not share with land-bound reptiles. A brain structure
adapted
to the demands of flight has occurred in evolutionary history long before
the appearance of birds. Pterosaurs, the first flying vertebrates,
show adaptations undergone by a reptilian-type brain in order to achieve
flight. Generally, much of the pterosaur brain
can be considered as intermediate between that of reptiles and birds.
Both birds and pterosaur brains exhibit proportionally large optic lobes,
small olfactory bulbs, and an expanded forebrain.
The optic lobes in Pterosaurs were similar
in position and proportions to that of modern birds. In
Pterodactylus, the optic lobes were very close to the orbit, so that the
optic nerve must have been extremely short (Edinger, 1941). Rhamphorynchus
and Pteronadon endocasts which represent only the upper half of the brain,
there are no discernible midbrain lobes.
These lobes have presumably descended, shifting basally from the reptilian
dorsal position (Edinger, 1941). In lateral views of Pterodactylus
specimens, the lobe chamber is well-defined and relatively large.
The optic lobes probably extended over the lower half of the brain in Pterodactylus,
and they were situated even further down in Rhamphorynchus. In the
later pterosaur, Pteranodon (Cretaceous), the optic lobes are confined
to the basal half of the brain or lower, a position similar to that in
modern birds (Edinger, 1941). The optic lobe size indicates that
they were highly developed, most likely in support of flight behaviors.
They were avian in character and very large compared to those of other
reptiles of the time. Some species of pterosaurs were small and probably
tree dwelling, while some were large, gliding sea birds. Pterosaurs
exhibited many adaptations for active flight, but many larger species were
probably gliders, such as the giant Quetzalcoatlus of the late Cretaceous,
with an estimated wingspan of 36-39 feet (11-12 meters), close to that
of a light aircraft (Wellnhofer, 1996).
Modern
birds - optic lobes. The optic tectum (TeO) in birds exhibits
characteristics important for motion processing. Approximately
90% of the retinal ganglion cell axons in lateral-eyed birds project to
the highly laminated TeO (Shimizu & Karten, 1991), a structure which
is likely homologous to the mammalian superior colliculus. The retinal
efferents to TeO travel the optic tract and terminate within the superficial
layers of the contralateral TeO (Karten & Revzin, 1966; Karten, 1969).
Most of the deep layers of TeO possess cells which exhibit large receptive
fields (70-180 degrees) and are motion-responsive and directionally selective
(Frost and DiFranco, 1976). Additionally, deeper tectal layers,
like the stratum griseum centrale (SGC) have cells which demonstrate a
high degree of habituation to repeated stimulus presentations (Woods and
Frost, 1977). The receptive-field size and response characteristics
are traits one would expect from a motion-processing area.
Pterosaurs
- cerebellum. The highly folded cerebellum ("little
brain") in all vertebrates is involved in the timing, coordination, and
modulation of the motor system with incoming visual, vestibular, auditory,
and somatosensory information. The cerebellum consists of two
basic divisions, the main body of the corpus cerebelli and a cerebellar
auricle ("little ear"). In general the vertebrate pattern consists of input
from primary or secondary nuclei of the cranial nerves and from a precerebellar
nucleus, the inferior olive. The output of the cerebellar cortex is to
one or more deep cerebellar nuclei which then project to the reticular
formation and the nucleus ruber (red nucleus). These two areas are important
sources of descending spinal pathways. A small caudal structure usually
on the cerebellum's ventrolateral aspect, the flocculus, receives input
from the vestibular system and sends output to motor neurons that control
the muscles involved in eye movements. The cerebellum also sends output
to other motor nuclei of the brainstem, portions of the thalamus involved
in motor functions, and vestibular nuclei.
The pterosaurian cerebella existed at
the upper limit of reptilian brains and the lower limit of avian variations (Edinger, 1941). The cranium of Pterodactylus elegans
had two incisures which were broad and hollow, corresponding to the tuber
cerebellare of modern birds (Edinger, 1941). In the same fossil
there was a "median dorsocaudal protuberance, delimited above and below
by transverse furrows", which Edinger states must represent the pterodactylian
cerebellum. Unfortunately, the cerebellar folds or gyri were not
impressed within the skull cavity, so much of its basic structure is speculative.
Edinger does note that descriptions of other pterosaur specimens indicate
the presence of flocculi.
Modern birds -
cerebellum. Many evolutionary changes seen in the
structural morphology of the cerebellum occurred with the transition of
aquatic forms to the terrestrial tetropods. With the development
of locomotor abilities and behaviors for grasping, digging, et cetera,
there was a concomitant lateral expansion and increase in folding.
Birds must have sophisticated cerebellar and vestibular systems to deal
with the complexities of movement in three dimensions. These systems must
work sufficiently to avoid obstacles, chase away encroachments on territories,
or even catch meals "on the wing." The bird's cerebellum is greatly
expanded and extensively folded in comparison to modern reptiles.
Avian and mammalian cerebella are large, highly folded structures which
have a very similar organization according to comparative studies. Birds
have 10 large folds or lobules in their cerebella. Mammals have 10 lobules
on the vermis, at the midline of the cerebellum, with many more lobules
on the lateral portions. This lateral expansion of the cerebellum
in mammals could be a phylogenetically new structure, but it is more likely
a variation on that seen in the other amniotes.
Pterosaurs
- forebrain. Pterosaurs (prehistoric flying reptiles)
achieved an "avian-like" forebrain long before the existence of birds.
The gross morphology and relative size of the forebrain, olfactory bulb,
and optic lobe associated with modern birds may have first evolved in the
prehistoric flying reptiles, the pterosaurs. In fact, Edinger (1941),
in a description of a Pterodactylus specimen (Pterodactylus elegans,
from about the late Jurassic), went so far as to say that the avian forebrain
form was achieved by the pterodactyloidea in the Cretaceous. The
modern bird forebrain is usually wider than it is long. Early pterosaurs
had brain proportions which were more reptilian, possessing a forebrain
that was longer than it was wide (Wellnhofer, 1996). This gradually
changed, with the Rhamphorhynchoidea (late Triassic and Early Jurassic)
exhibiting forebrain widths up to 1.5 times their length, a more "bird-like"
ratio. Pteranodon (early Cretaceous) forebrains reached similar proportions
to those of modern birds. The pterodactylus brain, as in birds, formed
an arch around the back of a relatively large eye orbit. Pterodactylus’
forebrain possessed two fissures similar to that of modern birds.
Pterodactylus and similar specimens show a horizontal furrow along the
middle portion of the forebrain which separates the upper and lower half
of the forebrain. This fissure would correspond to the avian valecula
(Edinger, 1941). A vertical furrow descending from the horizontal
fissure indicates the presence of a vallecula Sylvii, similar to that which
frontal and temporal sections of the modern bird’s basal forebrain.
Pterosaurs showed similar relative sizes
between olfactory bulbs and other aspects of the forebrain. At
the rostral end of the brain chamber in the pterosaur specimen described
by Edinger (1941), the tiny, tube-like chamber which housed the olfactory
bulbs was preserved. There were also no discernible olfactory tracts.
By comparison, in the Tuatara (Sphenodon punctatus), a large-eyed
reptile, the olfactory tracts may be almost as twice as long as the forebrain.
The olfactory bulb had about 1/25th the volume of the optic lobe; the Tuatara
has a 1:1 ratio of olfactory bulb to optic lobe. The ratios of olfactory
bulb to forebrain and optic lobe found in Pterodactylus are similar to
those found in modern birds. This strongly suggests that in pterosaurs,
as in modern birds, olfaction was not as prominent a sense as was the highly
developed eye and visual system.
Modern birds -
forebrain - nucleus rotundus. At the diencephalic level of the collothalamic
pathway, the nucleus rotundus (Rt) of the dorsal thalamus also exhibits
motion-processing regions. Neurons of the SGC layer of the optic
tectum send extensive projections to the largest single thalamic cell group
in many reptiles and birds, the nucleus rotundus (Benowitz & Karten,
1976; Ulinski, 1983). These projections are spatially organized,
with specific rotundal subdivisions receiving afferents from specific tectal
layers (Benowitz & Karten, 1976; Nixdorf & Bischof, 1982).
Approximately 80% of the neurons in Rt have very large receptive fields
(from 100 to 175°) and are motion-sensitive (Revzin, 1970). A
series of electophysiological experiments by Wang, Jiang, and Frost (1993)
indicated functional subdivisions for color, whole-field illumination levels,
and motion. The motion-sensitive neurons of Rt are found in higher
proportions in the posterior Rt (Wang, Jiang, and Frost, 1993). The
posterior, in turn, exhibits cells tuned either to motion in two dimensions
or that along the z-axis (motion in depth); (Wang et al., 1993).
Neurons in Rt send axons to the core ectostriatum (Karten & Hodos,
1970; Watanabe, Ito, & Ikushima, 1985).
Modern
birds - forebrain - ectostriatum. The primary telencephalic nucleus of
the collothalamic pathway, the ectostriatum, shows motion-processing characteristics
in birds. The response properties of ectostriatal neurons
show many similarities to those in Rt. Ectostriatal receptive fields
(150-180°) are similar to those found in Rt (100 to 175°); (Revzin,
1970). Ectostriatal cells typically respond best to upward/downward
or fore/aft movement with no selectivity for orientation (Revzin, 1970;
Kimberly et al., 1971). The ectostriatum in zebra finches also shows
a high degree of motion-responsiveness. Engelage
and Bischof (1996) found large receptive fields in ectostriatum, some of
which covered the entire visual field of the contralateral eye.
In addition, they performed a physiological analysis of ectostriatal neuron
firing rates upon exposure to various visual stimuli. Using bars
which could be moved in directions parallel or orthogonal to their longest
axis, Engelage and Bischof (1996) found about 80% of the neurons tested
were motion responsive. Of the units which reacted to bars moving
parallel to their longest axis, about 70% showed orientation-selectivity.
Conversely, of those neurons reacting to bars moving orthogonal to their
longest axis, only 37% showed orientation preferences. A sensitivity,
direction, and orientation analysis of ectostriatal neurons to sinusoidal
gratings also revealed slight preference for forward and upward movements.
There were also units which responded vigorously to "looming" stimuli (stimuli
moving towards the eye). The orientation selectivity of neurons which
responded to bars moving parallel to their longest axis could be indicative
of neurons which deal with optic flow in the environment during flight.
The somewhat higher sensitivity to backward movements may also be such
a specialization, and upward-movement sensitive neurons could serve as
tilt detectors during flight, as previous authors have suggested (Kimberly
et al., 1971; Engelage and Bischof, 1996). In addition, units which
showed high response rates to looming stimuli were similar to those of
TeO and Rt. These types of cells could theoretically be useful
in calculating "time to collision" (Wang and Frost, 1992; Wang et al.,
1993). The presence of significant proportions of motion-responsive
neurons in the ectostriatum, and at other levels of the collothalamic visual
pathway, indicates that a significant portion of the avian visual system
has developed to meet the perceptual demands inherent in the control of
flight behaviors. In addition, the ectostriatum has been shown to
project to several other telencephaic areas (Ritchie, 1979; Husband &
Shimizu, 1999), including the external pallium which sends significant
projections to the basal ganglia (Veenman et al., 1995).
Next Section: References and Links